Abstract
Quantifying environment–morphology relationships is important not only for understanding the fundamental processes driving phenotypic diversity within and among species but also for predicting how species will respond to ongoing global change. Despite a clear set of expectations motivated by ecological theory, broad evidence in support of generalizable effects of abiotic conditions on spatial and temporal intraspecific morphological variation has been limited. Using standardized data from >250,000 captures of 105 landbird species, we assessed intraspecific shifts in the morphology of adult male birds since 1989 while simultaneously measuring spatial morphological gradients across the North American continent. We found strong spatial and temporal trends in average body size, with warmer temperatures associated with smaller body sizes both at more equatorial latitudes and in more recent years. The magnitude of these thermal effects varied both across and within species, with results suggesting it is the warmest, rather than the coldest, temperatures that drive both spatial and temporal trends. Stronger responses to spatial—rather than temporal—variation in temperature suggest that morphological change may not be keeping up with the pace of climate change. Additionally, as elevation increases, we found that body size declines as relative wing length increases, probably due to the benefits that longer wings confer for flight in thin air environments. Our results provide support for both existing and new large-scale ecomorphological ‘rules’ and highlight how the response of functional trade-offs to abiotic variation drives morphological change.
This is a preview of subscription content, access via your institution
Access options
Access Nature and 54 other Nature Portfolio journals
Get Nature+, our best-value online-access subscription
$29.99 / 30 days
cancel any time
Subscribe to this journal
Receive 12 digital issues and online access to articles
$119.00 per year
only $9.92 per issue
Buy this article
- Purchase on Springer Link
- Instant access to full article PDF
Prices may be subject to local taxes which are calculated during checkout





Similar content being viewed by others
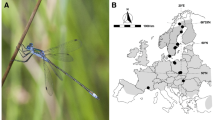
Data availability
Data from the MAPS programme are curated and managed by The Institute for Bird Populations and were queried from the MAPS database on 16 October 2019. MAPS data used here are available on Dryad (https://doi.org/10.5068/D1DT2T).
Code availability
All code used to produce analyses are freely available on Github (https://github.com/caseyyoungflesh/MAPS_morph_changes) and archived on Zenodo (https://doi.org/10.5281/zenodo.6977666).
References
Dehling, D. M., Jordano, P., Schaefer, H. M., Böhning-Gaese, K. & Schleuning, M. Morphology predicts species’ functional roles and their degree of specialization in plant–frugivore interactions. Proc. R. Soc. B 283, 20152444 (2016).
Grant, P. R. Inheritance of size and shape in a population of Darwin’s finches, Geospiza conirostris. Proc. R. Soc. Lond. B 220, 219–236 (1983).
Des Roches, S. et al. The ecological importance of intraspecific variation. Nat. Ecol. Evol. 2, 57–64 (2018).
Bergmann, C. Über die verhältnisse der wärmeökonomie der thiere zu ihrer grösse. Gött. Stud. 3, 595–708 (1847).
Allen, J. A. The influence of physical conditions in the genesis of species. Radic. Rev. 1, 108–140 (1877).
Altshuler, D. L. & Dudley, R. The physiology and biomechanics of avian flight at high altitude. Integr. Comp. Biol. 46, 62–71 (2006).
Teplitsky, C. & Millien, V. Climate warming and Bergmann’s rule through time: is there any evidence? Evol. Appl. 7, 156–168 (2014).
Gardner, J. L., Peters, A., Kearney, M. R., Joseph, L. & Heinsohn, R. Declining body size: a third universal response to warming? Trends Ecol. Evol. 26, 285–291 (2011).
Yom-Tov, Y., Yom-Tov, S., Wright, J., Thorne, C. J. R. & Du Feu, R. Recent changes in body weight and wing length among some British passerine birds. Oikos 112, 91–101 (2006).
Van Buskirk, J., Mulvihill, R. S. & Leberman, R. C. Declining body sizes in North American birds associated with climate change. Oikos 119, 1047–1055 (2010).
Weeks, B. C. et al. Shared morphological consequences of global warming in North American migratory birds. Ecol. Lett. 23, 316–325 (2020).
Rosenberg, K. V. et al. Decline of the North American avifauna. Science 366, 120–124 (2019).
DeSante, D. F., Saracco, J. F., O’Grady, D. R., Burton, K. M. & Walker, B. L. Methodological considerations of the Monitoring Avian Productivity and Survivorship (MAPS) program. Stud. Avian Biol. 29, 28–45 (2004).
West, G. B., Brown, J. H. & Enquist, B. J. A general model for the origin of allometric scaling laws in biology. Science 276, 122–126 (1997).
Jirinec, V. et al. Morphological consequences of climate change for resident birds in intact Amazonian rainforest. Sci. Adv. 7, eabk1743 (2021).
Dubiner, S. & Meiri, S. Widespread recent changes in morphology of Old World birds, global warming the immediate suspect. Glob. Ecol. Biogeogr. 31, 791–801 (2022).
Ballinger, M. A. & Nachman, M. W. The contribution of genetic and environmental effects to Bergmann’s rule and Allen’s rule in house mice. Am. Nat. https://doi.org/10.1086/719028 (2022).
Andrew, S. C., Hurley, L. L., Mariette, M. M. & Griffith, S. C. Higher temperatures during development reduce body size in the zebra finch in the laboratory and in the wild. J. Evol. Biol. 30, 2156–2164 (2017).
Siepielski, A. M. et al. No evidence that warmer temperatures are associated with selection for smaller body sizes. Proc. R. Soc. B 286, 20191332 (2019).
Salewski, V., Siebenrock, K.-H., Hochachka, W. M., Woog, F. & Fiedler, W. Morphological change to birds over 120 years is not explained by thermal adaptation to climate change. PLoS ONE 9, e101927 (2014).
Riddell, E. A., Iknayan, K. J., Wolf, B. O., Sinervo, B. & Beissinger, S. R. Cooling requirements fueled the collapse of a desert bird community from climate change. Proc. Natl Acad. Sci. USA 116, 21609–21615 (2019).
Pecl, G. T. et al. Biodiversity redistribution under climate change: impacts on ecosystems and human well-being. Science 355, eaai9214 (2017).
Futuyma, D. J. Evolutionary constraint and ecological consequences. Evolution 64, 1865–1884 (2010).
Murren, C. J. et al. Constraints on the evolution of phenotypic plasticity: limits and costs of phenotype and plasticity. Heredity 115, 293–301 (2015).
Rollinson, C. R. et al. Working across space and time: nonstationarity in ecological research and application. Front. Ecol. Environ. 19, 66–72 (2021).
Riemer, K., Guralnick, R. P. & White, E. P. No general relationship between mass and temperature in endothermic species. eLife 7, e27166 (2018).
Ryding, S., Klaassen, M., Tattersall, G. J., Gardner, J. L. & Symonds, M. R. Shape-shifting: changing animal morphologies as a response to climatic warming. Trends Ecol. Evol. 36, 1036–1048 (2021).
Baldwin, M. W., Winkler, H., Organ, C. L. & Helm, B. Wing pointedness associated with migratory distance in common-garden and comparative studies of stonechats (Saxicola torquata). J. Evol. Biol. 23, 1050–1063 (2010).
Förschler, M. I. & Bairlein, F. Morphological shifts of the external flight apparatus across the range of a passerine (Northern Wheatear) with diverging migratory behaviour. PLoS ONE 6, e18732 (2011).
Macpherson, M. P., Jahn, A. E. & Mason, N. A. Morphology of migration: associations between wing shape, bill morphology and migration in kingbirds (Tyrannus). Biol. J. Linn. Soc. 135, 71–83 (2022).
Newton, I. The Migration Ecology of Birds (Elsevier, 2010).
Clegg, S. M., Kelly, J. F., Kimura, M. & Smith, T. B. Combining genetic markers and stable isotopes to reveal population connectivity and migration patterns in a neotropical migrant, Wilson’s warbler (Wilsonia pusilla). Mol. Ecol. 12, 819–830 (2003).
Bell, C. P. Leap-frog migration in the fox sparrow: minimizing the cost of spring migration. Condor 99, 470–477 (1997).
Billerman, S., Keeney, B., Rodewald, P. & Schulenberg, T. (eds) Birds of the World (Cornell Laboratory of Ornithology, 2020).
Desrochers, A. Morphological response of songbirds to 100 years of landscape change in North America. Ecology 91, 1577–1582 (2010).
Swaddle, J. P. & Lockwood, R. Morphological adaptations to predation risk in passerines. J. Avian Biol. 29, 172–176 (1998).
Chown, S. L. & Klok, C. J. Altitudinal body size clines: latitudinal effects associated with changing seasonality. Ecography 26, 445–455 (2003).
Hsiung, A. C., Boyle, W. A., Cooper, R. J. & Chandler, R. B. Altitudinal migration: ecological drivers, knowledge gaps, and conservation implications: animal altitudinal migration review. Biol. Rev. 93, 2049–2070 (2018).
Barras, A. G., Liechti, F. & Arlettaz, R. Seasonal and daily movement patterns of an alpine passerine suggest high flexibility in relation to environmental conditions. J. Avian Biol. 52, jav.02860 (2021).
Spence, A. R. & Tingley, M. W. Body size and environment influence both intraspecific and interspecific variation in daily torpor use across hummingbirds. Funct. Ecol. 35, 870–883 (2021).
Moreau, R. E. Variation in the western Zosteropidae (Aves). Bull. Br. Mus. Nat. Hist. Zool. 4, 311–433 (1957).
Hamilton, T. H. The adaptive significances of intraspecific trends of variation in wing length and body size among bird species. Evolution 15, 180–194 (1961).
Hodkinson, I. D. Terrestrial insects along elevation gradients: species and community responses to altitude. Biol. Rev. 80, 489–513 (2005).
Feinsinger, P., Colwell, R. K., Terborgh, J. & Chaplin, S. B. Elevation and the morphology, flight energetics, and foraging ecology of tropical hummingbirds. Am. Nat. 113, 481–497 (1979).
Aldrich, J. W. Ecogeographical Variation in Size and Proportions of Song Sparrows (Melospiza melodia) (American Ornithological Society, 1984).
Sun, Y. et al. The role of climate factors in geographic variation in body mass and wing length in a passerine bird. Avian Res. 8, 1 (2017).
Des Roches, S., Pendleton, L. H., Shapiro, B. & Palkovacs, E. P. Conserving intraspecific variation for nature’s contributions to people. Nat. Ecol. Evol. 5, 574–582 (2021).
McKechnie, A. E. & Wolf, B. O. Climate change increases the likelihood of catastrophic avian mortality events during extreme heat waves. Biol. Lett. 6, 253–256 (2010).
Conradie, S. R., Woodborne, S. M., Cunningham, S. J. & McKechnie, A. E. Chronic, sublethal effects of high temperatures will cause severe declines in southern African arid-zone birds during the 21st century. Proc. Natl Acad. Sci. USA 116, 14065–14070 (2019).
Radchuk, V. et al. Adaptive responses of animals to climate change are most likely insufficient. Nat. Commun. 10, 3109 (2019).
Riddell, E. A. et al. Exposure to climate change drives stability or collapse of desert mammal and bird communities. Science 371, 633–636 (2021).
Tingley, M. W., Monahan, W. B., Beissinger, S. R. & Moritz, C. Birds track their Grinnellian niche through a century of climate change. Proc. Natl Acad. Sci. USA 106, 19637–19643 (2009).
Youngflesh, C. et al. Migratory strategy drives species-level variation in bird sensitivity to vegetation green-up. Nat. Ecol. Evol. 5, 987–994 (2021).
Blueweiss, L. et al. Relationships between body size and some life history parameters. Oecologia 37, 257–272 (1978).
Kleiber, M. Body size and metabolic rate. Physiol. Rev. 27, 511–541 (1947).
Yodzis, P. & Innes, S. Body size and consumer-resource dynamics. Am. Nat. 139, 1151–1175 (1992).
Prum, R. O. Interspecific social dominance mimicry in birds: social mimicry in birds. Zool. J. Linn. Soc. 172, 910–941 (2014).
Pyle, P. Identification Guide to North American Birds: A Compendium of Information on Identifying, Ageing, and Sexing ‘Near-Passerines’ and Passerines in the Hand (Slate Creek Press, 1997).
Leys, C., Ley, C., Klein, O., Bernard, P. & Licata, L. Detecting outliers: do not use standard deviation around the mean, use absolute deviation around the median. J. Exp. Soc. Psychol. 49, 764–766 (2013).
Danielson, J. J. & Gesch, D. B. Global Multi-Resolution Terrain Elevation Data 2010 (GMTED2010) (US Geological Survey, 2011).
Thornton, M. M. et al. Daymet: Daily Surface Weather Data on a 1-km Grid for North America, Version 4 (ORNL Distributed Active Archive Center, 2020).
Greenewalt, C. H. The flight of birds: the significant dimensions, their departure from the requirements for dimensional similarity, and the effect on flight aerodynamics of that departure. Trans. Am. Philos. Soc. 65, 1–67 (1975).
Longo, G. & Montévil, M. Perspectives on Organisms: Biological Time, Symmetries, and Singularities (Springer, 2014).
Harvey, P. H. in Scaling in Biology (eds Brown, J. H. & West, G. B.) 253–265 (Oxford Univ. Press, 2000).
Orme, D. et al. The caper package: comparative analysis of phylogenetics and evolution in R. R package version 5 (2013).
R Core Team. R: A Language and Environment for Statistical Computing (R Foundation for Statistical Computing, 2021).
Jetz, W., Thomas, G. H., Joy, J. B., Hartmann, K. & Mooers, A. O. The global diversity of birds in space and time. Nature 491, 444–448 (2012).
Nudds, R. L., Kaiser, G. W. & Dyke, G. J. Scaling of avian primary feather length. PLoS ONE 6, e15665 (2011).
Nudds, R. Wing-bone length allometry in birds. J. Avian Biol. 38, 515–519 (2007).
Anderson, S. C., Branch, T. A., Cooper, A. B. & Dulvy, N. K. Black-swan events in animal populations. Proc. Natl Acad. Sci. USA 114, 3252–3257 (2017).
Stan Modeling Language Users Guide and Reference Manual, Version 2.18.0 (Stan Development Team, 2018); http://mc-stan.org
Carpenter, B. et al. Stan: a probabilistic programming language. J. Stat. Softw. 76, 1–32 (2017).
Youngflesh, C. MCMCvis: tools to visualize, manipulate, and summarize MCMC output. J. Open Source Softw. 3, 640 (2018).
Wickham, H. et al. Welcome to the Tidyverse. J. Open Source Softw. 4, 1686 (2019).
Gabry, J., Simpson, D., Vehtari, A., Betancourt, M. & Gelman, A. Visualization in Bayesian workflow. J. R. Stat. Soc. A 182, 389–402 (2019).
McElreath, R. Statistical Rethinking: A Bayesian Course with Examples in R and Stan (Chapman and Hall/CRC, 2018).
Data Zone (BirdLife International, 2019); http://datazone.birdlife.org/species/requestdis
Cramp, S. & Brooks, D. Handbook of the Birds of Europe, the Middle East and North Africa. The Birds of the Western Palearctic, Vol. VI. Warblers (Oxford Univ. Press, 1992).
Che-Castaldo, J., Che-Castaldo, C. & Neel, M. C. Predictability of demographic rates based on phylogeny and biological similarity. Conserv. Biol. 32, 1290–1300 (2018).
Villemereuil, P., de, Wells, J. A., Edwards, R. D. & Blomberg, S. P. Bayesian models for comparative analysis integrating phylogenetic uncertainty. BMC Evol. Biol. 12, 102 (2012).
Revell, L. J. phytools: an R package for phylogenetic comparative biology (and other things). Methods Ecol. Evol. 3, 217–223 (2012).
Pagel, M. Inferring the historical patterns of biological evolution. Nature 401, 877–884 (1999).
Hendry, A. P. & Kinnison, M. T. Perspective: the pace of modern life: measuring rates of contemporary microevolution. Evolution 53, 1637–1653 (1999).
Gingerich, P. Rates of evolution: effects of time and temporal scaling. Science 222, 159–162 (1983).
Bird, J. P. et al. Generation lengths of the world’s birds and their implications for extinction risk. Conserv. Biol. 34, 1252–1261 (2020).
Gingerich, P. D. Rates of evolution. Annu. Rev. Ecol. Evol. Syst. 40, 657–675 (2009).
Bürger, R. & Lynch, M. Evolution and extinction in a changing environment: a quantitative-genetic analysis. Evolution 49, 151–163 (1995).
Hendry, A. P., Farrugia, T. J. & Kinnison, M. T. Human influences on rates of phenotypic change in wild animal populations. Mol. Ecol. 17, 20–29 (2008).
Acknowledgements
We thank MAPS station operators for collecting and sharing their data. D. Kaschube provided critical data access, assisted by R. Guralnick and R. LaFrance. We thank C. Che-Castaldo for helpful discussions regarding the statistical modelling. Illustrations were provided by L. Helton. Funding was provided by National Science Foundation grants EF 1703048 (M.W.T.) and EF 2033263 (M.W.T.).
Author information
Authors and Affiliations
Contributions
C.Y. led formal analysis. C.Y. and M.W.T. shared conceptualization and writing of the original draft. R.B.S. and J.F.S. facilitated data access. All authors contributed to review and editing of drafts.
Corresponding author
Ethics declarations
Competing interests
The authors declare no competing interests.
Peer review
Peer review information
Nature Ecology & Evolution thanks Nir Sapir and the other, anonymous, reviewer(s) for their contribution to the peer review of this work.
Additional information
Publisher’s note Springer Nature remains neutral with regard to jurisdictional claims in published maps and institutional affiliations.
Extended data
Extended Data Fig. 1 Morphological data availability over time.
Each horizontal line represents one of 1124 MAPS stations. Stations are ordered by latitude, from north (top) to south (bottom).
Extended Data Fig. 2 Derivation of morphological indices.
(A) Logged wing length as a function of logged mass for the 105 bird species considered in this study. Points represent mean values for each species. (B) The relationship between wing length (W) and mass (M) can be described by a power law, where c represents the scaling exponent. Logging both sides of the equation linearizes this model. Using a phylogenetic regression, c was estimated to be approximately 1/3 across species, as predicted by scaling theory. The negative arc tangent of this estimate (to convert the slope to radians) was used to create a rotation matrix. (C) For each species, the rotation matrix was used to reproject logged wing length and logged mass onto a new coordinate plane (top panel). Values for both the x and y axes were standardized to have a standard deviation of 1, to create a size index and wing index, representing the overall size of each individual bird and the degree to which wing length deviates from its expected value given the body mass of the individual, respectively (bottom panel).
Extended Data Fig. 3 Posterior estimates for (A) SI βIDX (Eq. 6), (B) SI γIDX (Eq. 11), and (C) SI θIDX (Eq. 11), denoting the change in size index for each species per 10 years, 10 degrees latitude, and 1000 m elevation, respectively.
Points represent the posterior medians, while thick and thin lines represent the 50% and 89% credible intervals, respectively. The dashed grey line represents zero in all cases.
Extended Data Fig. 4 Posterior estimates for (A) WI βIDX (Eq. 6), (B) WI γIDX (Eq. 11), and (C) WI θIDX (Eq. 11), denoting the change in wing index for each species per 10 years, 10 degrees latitude, and 1000 m elevation, respectively.
Points represent the posterior medians, while thick and thin lines represent the 50% and 89% credible intervals, respectively. The dashed grey line represents zero in all cases.
Extended Data Fig. 5 Posterior estimates for (A) Lag 0 γTVT (Eq. 15), (B) Lag 1 γTVT (Eq. 15), and (C) Lag 2 γTVT (Eq. 15), denoting the change in Size Index for each species per 1C change in temperature at lag 0, lag 1, lag 2, respectively (i.e., the effect of change in temperature over time).
Points represent the posterior medians, while thick and thin lines represent the 50% and 89% credible intervals, respectively. The dashed grey line represents zero in all cases.
Extended Data Fig. 6 Posterior estimates for βSVT (Eq. 19) denoting the change in size index for each species per 10 °C change in mean station temperature (i.e., the effect of change in temperature over space).
Points represent the posterior medians, while thick and thin lines represent the 50% and 89% credible intervals, respectively. The dashed grey line represents zero in all cases.
Extended Data Fig. 7 Posterior estimates for (A) \({\bf{\omega}}_{{\boldsymbol{ M}}_{{\mathbf{ TIME}}}}\) (Eq. 25), (B) \({\bf{\omega}}_{{\boldsymbol{M}}_{{\mathbf{LAT}}}}\) (Eq. 25), and (C) \({\bf{\omega}}_{{\boldsymbol{M}}_{{\mathbf{ELEV}}}}\) (Eq. 25), denoting the percent change in mass for each species over the 30-year study period, the latitudinal range across which each species was sampled, and the elevational range across which each species was sampled, respectively.
Points represent the posterior medians, while thick and thin lines represent the 50% and 89% credible intervals, respectively. The dashed grey line represents zero in all cases.
Extended Data Fig. 8 Posterior estimates for (A) \({\bf{\omega}}_{{\boldsymbol{ W}}_{{\mathbf{ TIME}}}}\) (Eq. 25), (B) \({\bf{\omega}}_{{\boldsymbol{W}}_{{\mathbf{LAT}}}}\) (Eq. 25), and (C) \({\bf{\omega}}_{{\boldsymbol{W}}_{{\mathbf{ELEV}}}}\) (Eq. 25), denoting the percent change in wing length for each species over the 30-year study period, the latitudinal range across which each species was sampled, and the elevational range across which each species was sampled, respectively.
Points represent the posterior medians, while thick and thin lines represent the 50% and 89% credible intervals, respectively. The dashed grey line represents zero in all cases.
Extended Data Fig. 9 Absolute value of the estimate rate of change (represented in units of haldanes [standard deviations per generation]) for body mass (|h|) for focal species in this study (red) and for species and traits presented in (79) (blue).
Traits considered by (79) varied by species and only species undergoing anthropogenic disturbance [as defined by (79)] were considered. The x-axis of the plot is truncated at 0.2 to facilitate visualization.
Extended Data Fig. 10 General trends (decrease in SI over time, increase in SI over latitude, and increase in WI over elevation) observed in this study as exhibited by Turdus migratorius (American robin).
(a) Observed size index measures at one MAPS banding station (located at 39.3°N, 84.8°W) plotted over time. (b) Observed size index measures plotted across latitude. (c) Observed wing index measures plotted across elevation. In all cases, each black point represents one individual, the posterior mean of the linear predictor is plotted in red, while the red ribbon represents the 89% CI.
Supplementary information
Supplementary Information
Supplementary Fig. 1 and Supplementary Tables 1–4.
Rights and permissions
Springer Nature or its licensor (e.g. a society or other partner) holds exclusive rights to this article under a publishing agreement with the author(s) or other rightsholder(s); author self-archiving of the accepted manuscript version of this article is solely governed by the terms of such publishing agreement and applicable law.
About this article
Cite this article
Youngflesh, C., Saracco, J.F., Siegel, R.B. et al. Abiotic conditions shape spatial and temporal morphological variation in North American birds. Nat Ecol Evol 6, 1860–1870 (2022). https://doi.org/10.1038/s41559-022-01893-x
Received:
Accepted:
Published:
Issue Date:
DOI: https://doi.org/10.1038/s41559-022-01893-x
This article is cited by
-
Reply to: Shrinking body size may not provide meaningful thermoregulatory benefits in a warmer world
Nature Ecology & Evolution (2024)
-
Shrinking body size may not provide meaningful thermoregulatory benefits in a warmer world
Nature Ecology & Evolution (2024)
-
Smaller birds with warmer temperatures
Nature Ecology & Evolution (2022)